The push and pull of life - bioelectronics
Electroactive polymers tune into the body’s electromechanical dialogue
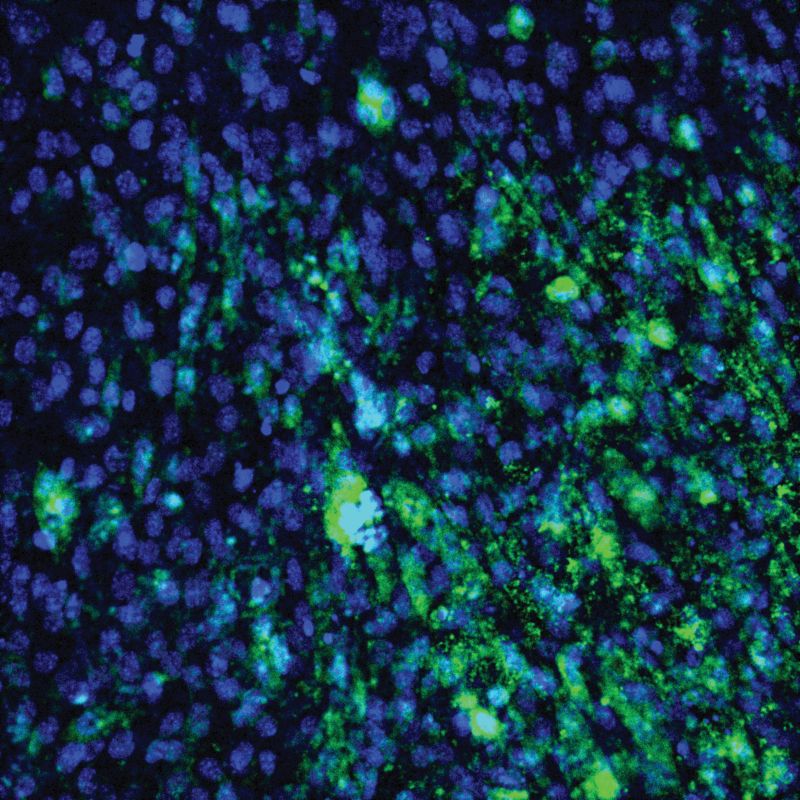
Life, like our modern world, is wired by electricity. Bioelectricity is a crucial element of the signalling in living systems, occurring within individual cells and in their organisation into complex tissues and organs.
With growing expertise in creating materials and devices that interact with electrical signals, there is a unique opportunity to develop biomedical materials that can probe, modulate, and monitor cell and tissue health.
Designing such materials for biomedical devices is not straightforward. In addition to ensuring that the materials can carry the electrical stimuli to and from cells, they must also be compatible for use in, or on, living tissues.
Biocompatibility is often used to describe the avoidance of harmful systemic effects, such as toxicity, irritation and sensitivity. However, this concept extends to providing the ideal match in mechanical and biochemical cues, necessary for the native tissue to be well-integrated with devices of interest, and to maintain the intended biological activity and performance. The biocompatibility of next-generation devices that satisfy these needs is inherently a materials challenge.
Activation energy
The tissues in our body are intrinsically receptive to electromechanical signals, extending from the superficial nerve endings lining the epidermal layer of the skin, right down to the highly attuned, light-sensitive, retinal rods and cones.
These nano- and microscale features allow us to visualise the changes in intensity and the spectrum of colours through alteration of the membrane potentials, which is transmitted through our optic nerve to the visual cortex. Within this complex bioelectric network, humans possess the exceptional capacity to distinguish and interpret letters and words inscribed on a page, enabling even the very act of reading this sentence.
Excitatory cells – such as neuronal, cardiac and muscular cells – along with their non-excitatory counterparts found in the skin, cartilage, bone and neoplastic cancers, all possess a quintessential resting electric potential. The electric potential difference across the cell membrane relies on the respective ion concentrations, which are regulated by ion channels on the membrane’s surface.
These include electrically and mechanically gated channels that open and close in response to the strain experienced by the surrounding cell. Consequently, all cells in the body exhibit sensitivity to electrical and mechanical stimuli, induced by alterations in ion flux within the extracellular matrix (ECM), or within the intracellular cytoskeleton.
In pursuit of deciphering this complex electromechanical dialogue, advancements in sensing and stimulating technologies aim to monitor and modulate tissue activity, paving the way for regenerative or therapeutic techniques.
Electroactive polymers (EAPs), a class of materials with the ability to deform under an applied electric field, are commonly used in soft robotics for artificial muscles, sensors and actuators. They have also recently emerged as a prime candidate to build smarter systems that can sense, modulate and integrate with human tissues while supporting fundamental biological function.
Much like our cells, electroactive materials are also capable of transducing electrical or mechanical input signals to achieve a controlled output response.
For instance, we can consider the behaviour of dielectric elastomers. These electronic EAPs undergo a dynamic transformation in shape when exposed to high voltage (kV) electric fields, leading to actuation of a membrane or the swelling and contraction of a construct.
Some EAPs, such as piezoelectric polymers, can also produce the converse effect by generating electrical outputs in response to mechanical stimuli, including shear, compression or tension. Subsequently, applications for dielectric elastomers are primarily found in actuators and sensors in artificial and soft robotic muscles.
These artificial muscles can flex and extend in a biomimetic manner, offering the potential for active control across multiple degrees of freedom, and increasing the range of functionality from the typical restrictions observed in single-plane robotic bodies.
Nevertheless, a persistent challenge that surrounds materials used in these minimally invasive and implantable devices is their lack of efficient degradability. This characteristic severely limits their integration with biological tissues for regenerative purposes.
Moreover, these materials are burdened with the need for exogenous input signals that are often inconsistent and can fluctuate drastically across the spectrum of physiological conditions, such as temperature, pH and humidity. Such variability can significantly impair their performance.
As we aim to leverage electromechanical signals in tissue engineering devices, it is essential to assess the effects of high voltages and strains on these delicate systems.
Adaptable tissue engineering devices can be created using low-voltage-driven materials, along with controlled strains through various ionic and electronic EAPs. These are used to explore the role of dynamic stimulation in growth, healing and as a protective measure against tumour tissues.
For instance, a 2023 article on Enhanced peripheral nerve regeneration by mechano-electrical stimulation, published in the journal npj Regenerative Medicine, demonstrates the mechano-electric effect of piezoelectric polymers in stimulating PC12 cells as a route to peripheral nerve regeneration.
There is potential for guided cell maturation, as well as enhanced neurite formation and extension on mechano-electric stimulated fibres, which has been reinforced by in vivo studies showing full axon reconnections in nerve gaps as large as 15mm.
On the other hand, conductive and electroactive cardiac patches have been recently investigated for their ability to interface with electrically excitable cardiac muscle cells following a cardiac event such as a myocardial infarction (MI), more commonly known as a heart attack.
The repercussions of an MI includes a reduction in the heart’s ability to pump blood efficiently around the body. This is partly due to the formation of scar tissue in the heart, alongside the loss of large populations of cardiomyocytes – the cells responsible for generating the heart’s contractile motion.
Cardiac patches have been developed to help restore functionality to the heart, supporting and regenerating the damaged tissue.
Electroactive materials are particularly promising in this respect, since they mimic the heart’s electrical conductivity and mechanical action. However, most commercially available cardiac patches and therapies to date include substrates that are neither conductive nor electroactive. This leaves scope for development.
One solution focuses on the development of electro/biological interfaces by using biologically derived materials as a non-toxic and inherently compatible substrate.
At the SMART Biomaterials Team at the Institute of Biomedical Engineering in Oxford, UK, we extend this approach by combining the soft robotic and sensing functionality offered by EAPs, with the biocompatibility of biopolymers derived from the native environment of tissues.
However, many ECM-derived biomaterials lack the necessary electromechanical functionality that allows synthetic interfaces to sense and stimulate tissue. By chemical conjugation of charged functional groups onto the backbone of ECM-delivered polymers, we can develop materials that are capable of tuneable mechanical deformation when subject to an electric field.
These systems – termed ionic ECMs – represent an initial step towards developing fully bioresorbable platforms, capable of providing electromechanical stimulation within a biologically representative substrate.
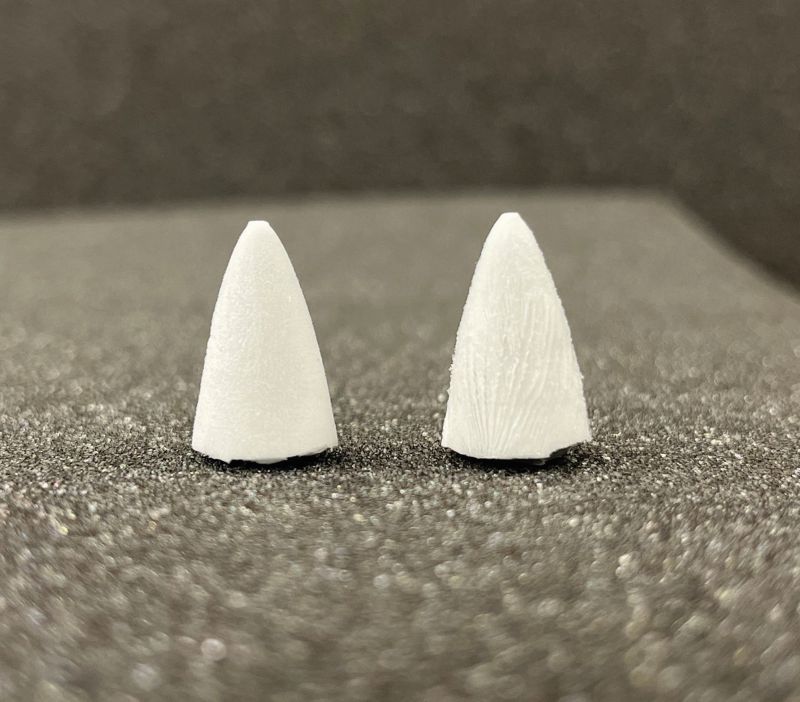
In addressing the foremost challenges in tissue engineering and regenerative medicine, electroactive biomaterials offer potential for use in a variety of areas of biomedical engineering.
Using dynamic, electroactive, cardiac scaffolds, we replicate the aligned microstructure of the heart fibres and the collagen-rich ECM to mimic the mechanical and structural cues found in the healthy heart. This is while capitalising on the ability to generate forces and strains under an applied field, to both stimulate existing populations of cardiomyocytes and restore the contractile function to the compromised organ.
Real-time adjustments
In electroactive biomaterials, systems that can manage and regulate material behaviour are required to effectively enable actuation and sensing performance. Depending on the application, control systems are critical in handling stimuli to the device and measuring the material’s response.
In many cases, devices are developed that regulate the input stimuli using the feedback gained from the sensing data – known as closed-loop control – whereas those that do not do this are known as open-loop control systems.
Integrating closed-loop control has multiple benefits, such as improving device efficiency, efficacy and safety. The most well-known example includes cardiac pacemakers used to send electrical pulses to the heart to ensure that it continues to beat with a regular rhythm. However, increasingly there are more devices that aim to provide controlled drug delivery by monitoring the patient’s condition and then triggering the release of drugs when necessary.
The major clinical example of this is closed-loop insulin delivery systems for patients with type 1 diabetes. These systems stabilise the patient’s blood sugar levels while reducing the number of blood tests required, thus improving the patient’s quality of life.
Similarly, systems that directly monitor cell and tissue conditions have also been extensively investigated for use in research and clinical settings.
A silicon-based device presented in the article Materials and device designs for wireless monitoring of temperature and thermal transport properties of wound beds during healing, published in the journal Advanced healthcare materials, measured temperature at wound sites to help caregivers treat patients with chronic wounds.
For biomaterials that can provide electromechanical stimuli to cells or tissues, closed-loop systems can enable real-time tuneability of those stimulation parameters. This is important for applications where both tissue regeneration and biodegradation are present, as the conditions experienced by the cells are subject to an ever-changing environment and the structure of the biomaterial itself is changed through cellular remodelling and degradation.
A closed-loop control system would enable the device to optimise the stimulation inputs in real-time throughout its operational lifespan, providing better treatment over a longer time frame.
Additionally, the sensing element used in the controller feedback would provide users with real-time updates on the device’s performance. An example of this is already seen in most clinical pacemakers, where there is an additional sensor that accounts for body movement and breathing rate separately to sensing the heart rate. This ensures that only the desired signals are used to assess when the device should intervene.
Working autonomously?
An ideal closed-loop controlled device for therapeutic delivery would be an autonomous system. Fully autonomous control would enable the device to make a decision about which stimuli/input to provide to the system without the input of humans.
Most systems employed in current clinical practice are semi-autonomous due to safety and regulatory concerns around a fully automatic device. This means that an expert or informed human, such as the surgeon implanting the device or the clinician reviewing the performance, has some level of input into the device’s decisions.
For example, the closed-loop-controlled, insulin-delivery system still requires the patient to perform blood tests at a significantly reduced rate compared to living without the device.
For manufacturers, creating fully autonomous devices is disincentivised by the higher level of regulation placed on them, as both the European Medicines Agency and US Food and Drug Administration categorise them as class III medical devices.
Consequently, the higher regulations and clinical testing requirements increase the time to market and the cost of development. Most manufacturers therefore opt for semi-autonomous designs where human input is still required.
Ensuring the correct balance is struck between maintaining user safety standards and fostering innovation is important across all commercial landscapes. This is particularly the case regarding novel biomaterials and their control systems due to the high level of risk and benefits the technology poses.
While there have been significant advances in this area, several challenges remain in achieving the ideal electroactive materials that can combine, sensing, stimulating and regenerative capabilities with or without autonomous control.
For instance, there is a strong need for the development of a wider range of materials that allow for cells to attach and grow as they would naturally within different tissues. Significant progress has been made on this front both within the UK and globally through the modification of natural materials and biocompatible synthetic materials.
However, this cannot be done without enhancing the ability to conduct and store electrical charges or ions, to support the conversion of energy from one form (mechanical or electric) to another. By combining the two functionalities, there is therefore significant scope for a more connected future, as we gain the ability to read and guide the electrical signatures of our tissue into better health.