Quantum leap for quantum technologies
A deep dive into materials science for quantum technologies.
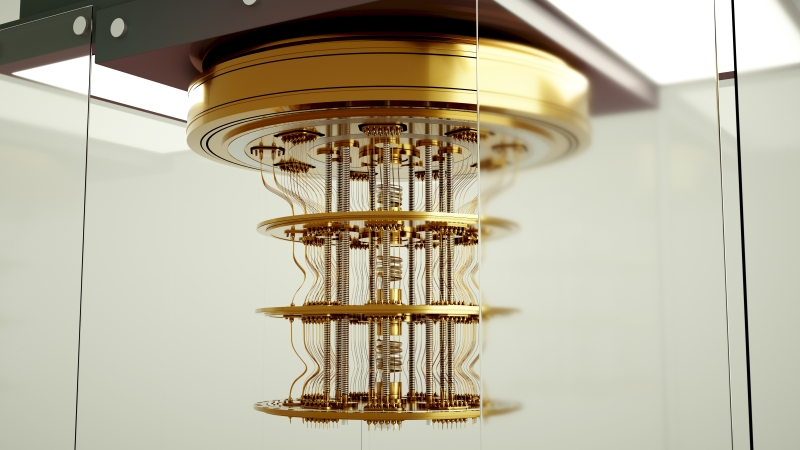
Quantum technologies are heralded as a revolutionary new paradigm for computing, sensing and communications. This stems from using quantum mechanical principles – including coherence, entanglement and superposition (see box-out below) – to surpass classical device limitations.
A much-publicised subset of quantum technologies is quantum computing, which aims to leverage arrays of quantum systems to do computation and simulation.
As opposed to classical computing that manipulates data in the form of bits representing 0s or 1s, quantum computing uses quantum bits, or ‘qubits’, based on superpositions that represent combinations of 0s and 1s simultaneously. These computers scale in such a way that each additional qubit doubles the number of states that can be stored concurrently.
This radical difference translates to enormous computing power at relatively small numbers of qubits – potentially tens of thousands compared to billions of conventional transistors. This offers unparalleled information densities that could tackle otherwise intractable calculations.
Defining the phenomenal
The smallest information unit in a computer is a bit and is binary – either 0/1. A quantum bit (qubit) is the smallest unit of information in a quantum computer and can represent a combination of 0 and 1 simultaneously.When measuring a qubit, it can only be seen in one of two states, but before the measurement is taken, it is in a superposition of both states. This phenomenon of superposition arises from quantum mechanics.
Quantum coherence deals with the idea that particles – photons, electrons or atoms – have wave-like properties, and superpositions result from the coherent interference of these waves.
Quantum entanglement sees a mixture of states extending over more than one qubit, such that each particle’s state can’t be described independently of the other. Measurement outcomes on one qubit are correlated to the other, even if separated by a distance.
The potential applications are bounded only by our imagination, and quantum computing has been proposed for drug design, materials discovery, weather prediction, traffic management and finance.
As quantum mechanical wavefunctions are inherently probabilistic, special quantum algorithms are needed to manipulate and use data that is generated by chance outcomes. Two famous examples are Shor’s algorithm for factorisation and Grover’s algorithm for rapid searches of unsorted databases.
Although, the implications of quantum computing undermining current encryption protocols is a major concern for national security. In response to this, quantum-secure communication has emerged as another branch of the technology and is a major driver for government investment.
United at the atom
However, realising these technologies is proving to be a titanic undertaking requiring a coordinated effort across many disciplines, including physics, electronics engineering, materials science and quantum information theory – itself an interdisciplinary field of mathematics, quantum mechanics, computer science, cryptography and others.
With so much at stake, the concerted international effort has developed into a fast-moving field. According to a Quanta Magazine article, quantum computing power is following a doubly exponential growth – one of the few real-world examples of this – underlining an imminent gain relative to classical computing.
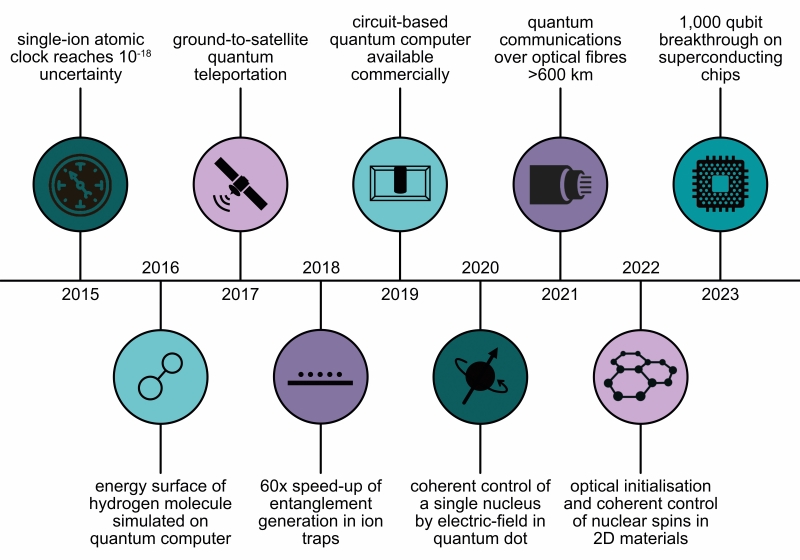
Plugging away
Quantum computing chips containing thousands of qubits have been made, although the current regime of low fidelities renders them ‘noisy’ and requiring error correction.
The number of ‘logical’ qubits in a single device reached 140 last year. Some of the calculations demonstrated at this scale include simulations of lattice models for condensed matter physics, calculations of ground-state energies of small molecules, numerical solvers and combinatorial optimisation problems.
Ahead of us remains the hallowed milestone of ‘quantum advantage’ – the point at which a quantum processor outperforms the most powerful conventional supercomputers. Several claims of this have been made and contested, and so the timeline for reaching quantum advantage remains uncertain.
Beyond the hype of quantum computing, quantum sensing is quietly developing with significant promise. These devices employ entangled sources and quantum measurements to beat classical limits, with the field providing some of the most mature quantum devices. Superconducting quantum interference device (SQUID) magnetometers are in use as the most sensitive magnetic field detectors, atomic clocks are setting the universal standards for timekeeping, and nitrogen-vacancy centres in diamonds are emerging in nanoscale imaging applications.
Within quantum communications, prototype networks have successfully sent entangled photons down fibre optic cables. These have achieved quantum entanglement between devices separated by kilometres, with quantum key distribution (a secure communication method) reaching over 1,000km, and quantum teleportation to three separate locations.
Despite these successes, quantum technologies are a way off from achieving their full potential. Step changes are needed to get large-scale quantum networks in place.
Quantum computing has arguably not demonstrated any advantage over conventional computing, while practical quantum advantage is even further out of reach. At the heart of this challenge is the nature of quantum systems, which are inherently fragile.
In the system
In general, quantum systems have discrete, resolvable energy levels. We are usually concerned with two-level systems that can be initialised into a desired state and have a final state read-out. There is often the need to coherently manipulate the state before read-out.
With these broad criteria, there are many physical platforms that fit the bill. Popular choices include quantum dots, point defects in semiconductors, photons, ion traps, neutral atoms, superconducting Josephson junctions and molecules. Quasiparticles in topological materials are another notable proposal, although they remain experimentally elusive.
Individual applications determine how these quantum systems are implemented and built into devices. For instance, quantum sensors can be used as ensembles or individual qubits and may require close proximity to surfaces. Quantum computers require large arrays with a degree of connectivity between individual qubits. A large-scale quantum communications network will transmit photons as ‘flying qubits’, but requires interfacing at node points for emission and collection, as well as quantum repeater units along the route.
As it stands, the prevailing hardware for quantum technologies remains an open question. With such a range of devices in the ecosystem, a combination of platforms will likely be in use in the years to come. Furthermore, the choice of current technologies is not set in stone and there is room for novel quantum systems that may provide different advantages.
Close control
With the aim of precise control over the system’s behaviour, this naturally requires the materials composing and containing it to be finessed.
The overarching challenge is the balance of coherence and control – to obtain high fidelities, a large number of operations must be performed before the system loses coherence. So, either the time taken to carry out each operation must be reduced, or the coherence time must be increased. This plays out as a balancing act between isolating the quantum system from environmental disturbances and leaving a route open for controlled manipulation.
This manifests as a series of hurdles in each materials platform, for instance removing ever-diminishing sources of ‘noise’. Such materials challenges are foreseen beyond the currently known bottlenecks, as many orders of magnitude improvements in fidelity are needed to move beyond the current ‘noisy intermediate-scale’ era of quantum computing (see box-out below).
While the precise origins of ‘noise’ are often unknown, the chemistries of surfaces and interfaces are recurring culprits. Shallow point-defect-based qubits are affected by surface noise, limiting their use as sensors and in the manufacture of arrays. In trapped ion systems, the trap electrodes, consisting of gold film, are a potential source of electromagnetic fluctuations.
Superconducting and quantum dot qubits
Superconducting qubits provide some of the largest quantum computing chips, reaching over 1,000 qubits, and are backed by research from IBM, Google and Intel.Qubit states are chosen as distinct physical states of a device built from Josephson junctions – a tiny electrical component formed by two ends of a superconducting
wire separated by a thin layer of insulator.
To achieve high fidelities, each superconducting qubit must act identically, as an artificial atom. However, being a component made up of many thousands of atoms, nanoscale variations are unavoidable.
To minimise these discrepancies, the superconducting materials used are single elements, commonly niobium as it has the highest superconducting transition temperature among metals of -263.95°C.
A native oxide layer forms on the surface of niobium, giving rise to magnetic impurities and limiting the superconducting properties. These oxide layers are often amorphous, making their characterisation challenging. Despite extensive research on the mechanisms of decoherence and improvements in surface treatment and fabrication, the microscopic origins of noise in superconducting systems are unknown. Nonetheless, the limit to the performance of these qubits likely stems from an uncontrolled surface chemistry.
One simple idea to mitigate these losses was to replace niobium with tantalum. This metal has a much simpler stoichiometry of oxides than niobium and forms an insulating oxide of Ta2O5, in contrast to non-insulating species arising in NbO, NbO2 and Nb2O5.
This successful adaptation results in longer coherence times, beating the previous state-of-the-art with consistency across devices. This is an example of how a reasoned choice of material can improve reproducibility and therefore overall performance of a quantum device.
However, there are still many avenues to explore in the hunt for microscopic sources of noise – grain size and interface quality being two that might prompt further interest from materials scientists.
A second example of materials solutions to decoherence is in the progress of quantum dot qubits. In this platform, individual electron spins from donors (e.g. phosphorus) in single-crystal silicon are placed with atomic precision and controlled by surrounding electrical contacts.
Advantages include the small on-chip footprint and compatibility with semiconductor manufacturing technology, giving a potential route to scale up. Over two decades of work has gone into developing these systems, including advances in epitaxial interfaces and isotopic purification to minimise intrinsic sources of charge and spin fluctuations.
More broadly, the issue of noise sources and other manufacturing and engineering challenges relate to the whole apparatus, extending to auxiliary components, and materials solutions will be at the heart of many of these.
Systematic improvements in materials selection and processing is a well-established strategy in many industries, but only in the past few years has this been taken as an approach to improve quantum technologies. This is likely because fabrication and control systems have developed to the extent that materials-based losses are now a primary constraint in achieving the coherence times required for applications.
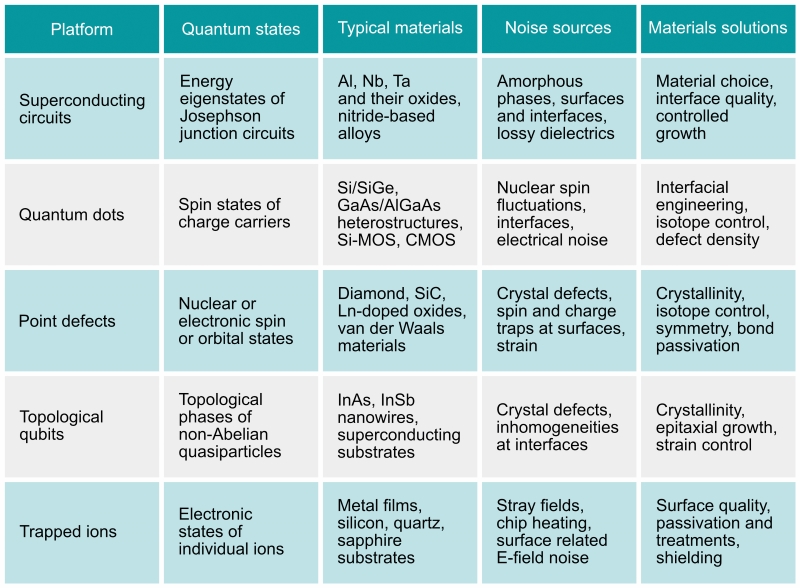
We are now at the point where materials improvements can have a marked effect on quantum device performance, with an understanding of the materials’ influence opening rational paths for progression. As well as the materials questions underlying these relatively mature technologies, there is space for new concepts to be established – where novel routes to control, coupling and scalability may all emerge from a materials basis (see table above).
Between theory and practice
There is an untapped wealth of expertise in the materials community that could help progress this quantum revolution. Materials scientists are needed to aid in materials selection, uncover new couplings and phenomena, and minimise sources of noise.
Considering the range of hardware in contention, scientists from nanomaterials, ceramics, metals, surface science, crystallography, electronics and magnetism fields will find an abundance of unanswered questions requiring their expertise.
As these technologies become incorporated into more systems, the fields of biomaterials, composites and manufacturing will find increasing overlap with quantum.
Both theoreticians and experimentalists are essential, especially for the correct interpretation of phenomena in emerging materials systems. For instance, there are many examples of point-defect systems that were predicted to be viable qubits several years before being validated in experiments. The strength of the UK’s computational materials chemistry community could be a major asset in this regard.
The UK Government has a 10-year strategy to ensure a quantum-enabled and quantum-secure society. However, we are still at an early stage of technological readiness, with the canvas for designing practical quantum technology remaining open.
At this design stage, the input of end-users is vital to steer the technology to a useful output. Unlike the mature platforms of classical computing that enable users to be system agnostic, the first to benefit from quantum computing will have worked closely with its algorithm developers to identify problems in their field that are well-suited to near-term quantum resources.
The full supporting infrastructure can then be built up of tailored quantum circuits, judicious choice of hardware, classical benchmarking and potentially hybrid, quantum-classical, computing integration. As such, early adopters will be able to influence development towards practical advantage within their field.
Some of the early targets for quantum computing and sensing are in the realm of materials science research and related areas of physics, chemistry and life sciences. Materials scientists are in the enviable position of being both needed to realise quantum technologies and benefitting as end-users. By getting involved at this stage, we can lend our expertise to the collective effort, shape these exciting technologies and reap the benefits for the field.